Unified View of High-Tc Superconductivity and Quantum Spin Liquids Revealed by Ab initio Supercomputer Simulations
PI of Joint-use project: M. Imada
Host lab: Supercomputer Center
Host lab: Supercomputer Center
Since the discovery of superconductivity in 1986 in copper oxides with quasi-two-dimensional perovskite structure, more than 35 years have already passed. However, its mechanism has not converged to consensus in the community because of the difficulty in treating strongly correlated electron systems, to which the cuprates belong.
Recently, numerical methods to study the strongly correlated electron systems have largely been developed [1], in which effective Hamiltonians derived by an ab initio framework starting from given crystal structure without resorting to adjustable parameters are solved by accurate quantum many-body solvers. The solutions of effective Hamiltonians for a number of copper oxide compounds correctly reproduce d-wave superconducting ground states [2,3]. From the relation between the ab initio parameters and the obtained superconducting order parameters, the principal component that controls the strength of the superconductivity was found. The results further show that the diverse materials dependence is well captured as shown in Fig. 1 for the superconducting critical temperature Tc. It was also clarified how the superconductivity can be enhanced in the present mechanism beyond the existing materials.
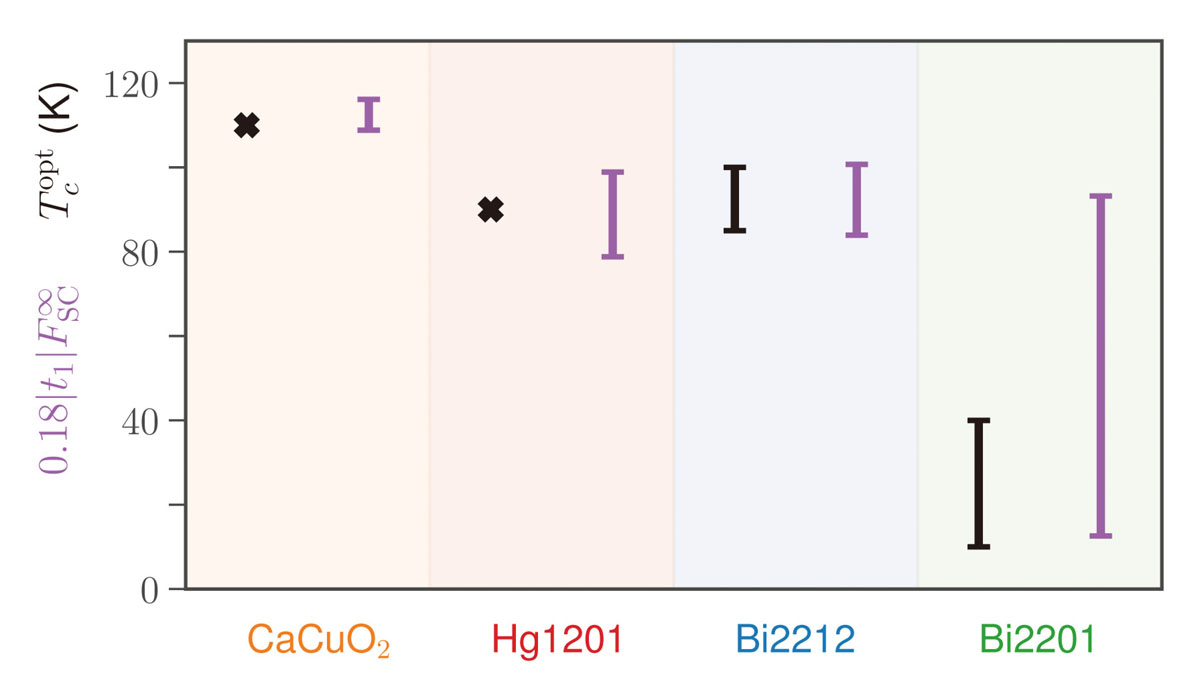
Fig. 1. Comparison of experimentally optimal critical temperature, Tcopt (black symbols) and the scaling formula 0.16 t1FSC∞ (purple symbols) obtained from the ab initio parameter t1 (nearest neighbor electron transfer) and the calculated order parameter FSC∞ [3]. Calculated results reproduce experimental trend of Tc quantitatively for carrier doped CaCuO2, HgBa2CuO4 (Hg1201), Bi2Sr2CaCu2O8 (Bi2212), and Bi2Sr2CuO6 (Bi2201) .
The ab initio solution also offers insights into electron fractionalization. It was found that an electron that is an elementary particle in vacuum is emergently splintered in strongly correlated electron systems. Thus generated particles are mutually tunneling quantum mechanically, in other words, hybridizing each other. The fractionalization well accounts for spectroscopic data otherwise puzzling, by combining experimental and computational results with the help of machine learning in the analyses of angle resolved photoemission and the resonant inelastic X-ray scattering results [4-7].
Another challenge of condensed matter physics is the nature of quantum fluids, especially, the quantum spin liquid since the first proposal half a century ago [8]. It was shown with the aid of supercomputers that the quantum spin liquid phase indeed exists and the spin excitations are well described by the fractionalized spinons in gapless quantum spin liquids, where the spinon has the Dirac-type gapless excitation and an observable spin excitation is represented by the two composite excitations of the spinon [9,10]. Ab initio calculations of molecular solid called dmit salts indeed demonstrated that this picture holds and the experimental phase diagram of the dmit salts is reproduced [10] as shown in Fig. 2 and the quantum spin liquid established in a theoretical touchstone model, J1-J2 Heisenberg Hamiltonian on the square lattice also shows essentially the same structure of the fractionalized excitation [9]. These findings were made it possible by the progress in quantum many-body solver using the neural network [11].
The electron fractionalization found in the cuprate superconductors and the fractionalization of an electronic spin into two spinons look quite different at a glance. However, the essence of the superconducting state as well as the quantum spin liquid lies in the way of constructing quantum mechanically entangled state, the element of which is commonly the paired state of two electrons with further entanglement of the pairs. Indeed, the wavefunctions have a common structure of the pair-product functions at the core. The quantum entanglement by the spin singlet constituting the quantum spin liquid and by the exciton leading to the electron fractionalization are represented in the extended Hilbert space in a unified fashion and the singlet and exciton take on different aspect of the same entanglement [4].
Acknowledgements
This report is based on the collaborations with Michael Schmid, Jean-Baptiste Morèe, Youhei Yamaji, Motoaki Hirayama, Yusuke Nomura, Di-Jing Huang, Atsushi Fujimori, Teppei Yoshida, Kota Ido, Takahiro Misawa, and Kazuyoshi Yoshimi.
References
- [1] M. Imada and T. Miyake, J. Phys. Soc. Jpn. 79, 112001 (2010).
- [2] J.-B. Morèe, M. Hirayama, M. T. Schmid, Y. Yamaji, and M. Imada, Phys. Rev. B 106, 235150 (2022).
- [3] M. T. Schmid, J.-B. Morèe, Y. Yamaji, and M. Imada, arXiv:2303.06672.
- [4] M. Imada, J. Phys. Soc. Jpn. 90, 111009 (2021).
- [5] M. Imada, J. Phys. Soc. Jpn. 90, 074702 (2021).
- [6] A. Singh, H. Y. Huang, J. D. Xie, J. Okamoto, C. T. Chen, T. Watanabe, A. Fujimori, M. Imada, D. J. Huang, Nat. Commun. 13, 7906 (2022).
- [7] Y. Yamaji, T. Yoshida, A. Fujimori, M. Imada, Phys. Rev. Research 3, 043099 (2021).
- [8] P. Fazekas and P. W. Anderson, Phil. Mag. 30, 423 (1974).
- [9] Y. Nomura and M. Imada, Phys. Rev. X 11, 031034 (2021).
- [10] K. Ido, K. Yoshimi, T. Misawa, and M. Imada, npj Quantum Mater. 7, 48 (2022).
- [11] Y. Nomura, A. S. Darmawan, Y. Yamaji and M. Imada, Phys. Rev. B 96, 205152 (2017).