Spin Dynamics in Equilateral Triangular Spin Tube Material CsCrF4
Masuda Group
Frustrated magnetism has attracted significant attention due to their potential to exhibit unique magnetic phases resulting from the competition between various interactions. Lattices composed of triangles as basic units, such as the triangular lattice, Kagome lattice, and Pyrochlore lattice, serve as representative examples of frustrated magnet systems. Triangular spin tube has been extensively studied theoretically as a one-dimensional frustrated system, where triangles are arranged in a single direction. In the Heisenberg equilateral triangular spin tube system, the spin correlation decays exponentially, and the ground state is a dimerized nonmagnetic state with a spin gap [1]. Furthermore, the presence of a Tomonaga-Luttinger liquid with chiral order has been predicted under the influence of anisotropy and a magnetic field [2].
In real materials, the presence of intertube interactions, albeit small, leads to the emergence of various magnetically ordered states [3]. As shown in Fig. 1(a), CsCrF4 consists of Cr3+ ions forming equilateral triangular spin tubes with S = 3/2 spins. Magnetic susceptibility and heat capacity measurements did not exhibit any sign of phase transitions [4]. Nevertheless, neutron diffraction experiments unveiled the presence of a long-range order characterized by a 120-degree structure, along with successive phase transitions where the propagation vector changes from qLT in Fig. 1(b) to qIT in Fig. 1(c) [5]. So far, the spin dynamics of this material have remained unexplored. In the present research, inelastic neutron scattering (INS) experiments were conducted using powder samples to determine the spin Hamiltonian and shed light on the successive phase transitions.
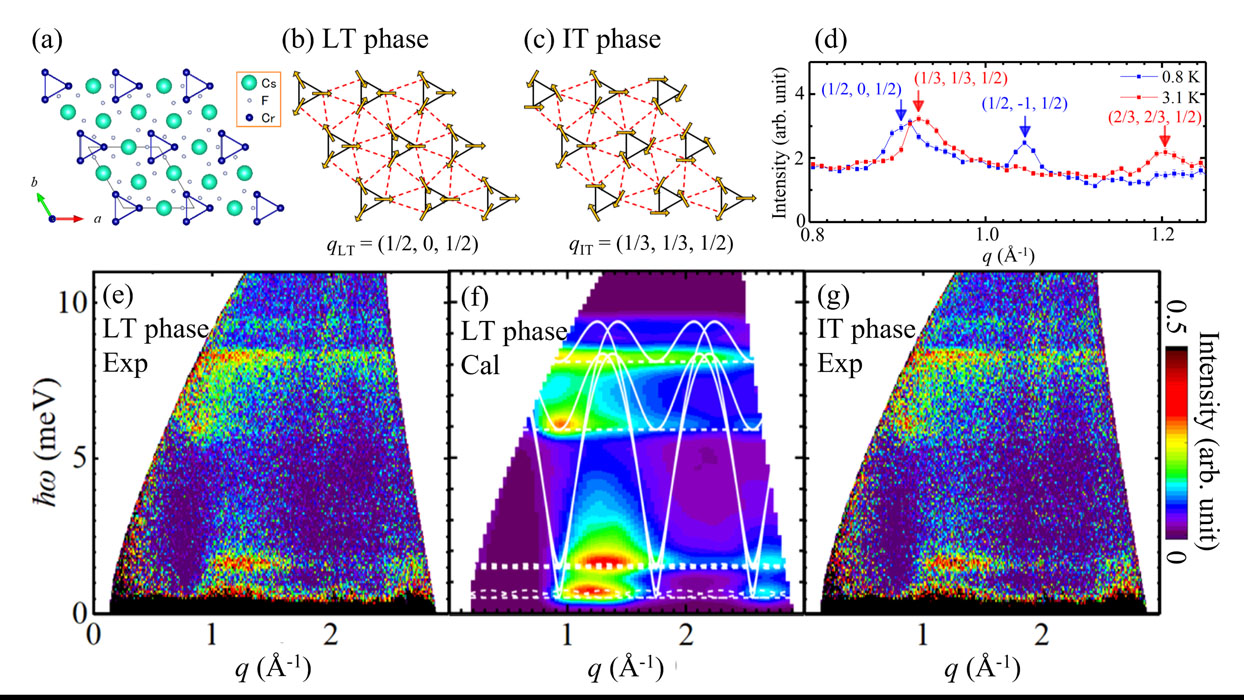
Fig. 1.(a) Crystal structure of CsCrF4. Cr ions form equilateral triangular spin tubes with S = 3/2 spins along the crystallographic c axis. (b) Magnetic structure of CsCrF4 in the LT phase. (c) Magnetic structure of CsCrF4 in the IT phase. (d) Elastic part of INS spectra measured with Ei = 3.1 meV. (e) INS spectra measured with Ei = 15.3 meV at 0.8 K (LT phase). (f) Powder averaged INS spectra in LT phase calculated by linear spin-wave theory. The white solid and dashed curves are dispersion relations calculated along the c* and a* axes, respectively. (g) INS spectra measured with Ei = 15.3 meV at 3.1 K (IT phase).
The experiment was conducted using the HRC spectrometer installed at J-PARC/MLF [6]. As shown in Fig. 1(d), magnetic Bragg peaks with qLT were observed at 0.8 K and those with qIT were observed at 3.1 K in the elastic part of the neutron spectra [5]. The observed and calculated INS spectra in the LT phase are shown in Figs. 1(e) and 1(f), respectively. For the latter, we used linear spin-wave theory on the basis of Heisenberg model of a triangular spin tube, including small DM interaction and a small single-ion anisotropy. The experiment was reasonably reproduced by the calculation. The spectrum in wide-energy range is explained by the quasi-one-dimensional nature of the spin tube in the c* direction. The analysis highlighted the significant roles of the DM interaction and single-ion anisotropy in low-energy range.
Furthermore, as depicted in Fig. 1(g), the INS spectrum in the IT phase was measured. Surprisingly, despite the change in the propagation vector in the diffraction profiles in Fig. 1(d), the INS spectrum in the IT phase exhibited a striking resemblance to the LT phase in level of powder-averaged spectra. The same analysis method utilized for the LT phase was applied to analyze the INS spectrum in the IT phase. However, the INS spectra in the IT phase could not be reproduced, indicating that the successive phase transition is not driven by variations in the spin Hamiltonian parameters.
To gain further insights, a phase diagram of the ground state was calculated for the coupled spin tubes system. The parameter values of the spin Hamiltonian estimated by the present INS experiment lie near the boundary between the magnetic phases with the structures of qIT and qLT. This finding implies that the internal energy of the magnetic structures in the LT and IT phases is similar. Considering that large spin entropy can favor the selection of the magnetic structure, it is proposed that the gain in spin entropy serves as the origin of the successive phase transition. Notably, this study provides the first evidence that, despite disparities in the static structures, the dynamic structures exhibit similarity under such circumstances.
References
- [1] K. Kawano, and M. Takahashi, J. Phys. Soc. Jpn. 66, 4001 (1997).
- [2] T. Sakai, M. Sato, K. Okunishi, Y. Otsuka, K. Okamoto, and C. Itoi, Phys. Rev. B 78, 184415 (2008).
- [3] K. Seki and K. Okunishi, Phys. Rev. B 91, 224403 (2015).
- [4] H. Manaka, Y. Hirai, Y. Hachigo, M. Mitsunaga, M. Ito, and N. Terada, J. Phys. Soc. Jpn. 78, 093701 (2009).
- [5] M. Hagihala, S. Hayashida, M. Avdeev, H. Manaka, H. Kikuchi, and T. Masuda, npj Quantum Mater. 4, 14 (2019).
- [6] H. Kikuchi, S. Asai, H. Manaka, M. Hagihala, S. Itoh, and T. Masuda, Phys. Rev. B 107, 184404 (2023).