Fabrication of a Compact Cubic-Anvil Pressure cell
N. Fujiwara and Y. Uwatoko
We have succeeded in fabricating the smallest cubic-anvil pressure cell (CAPC) to date. This miniaturization allowed us to investigate physical properties at high pressures of over 4 GPa under a magnetic field [1, 2].
In strongly correlated electron systems, one of the most intriguing topics is the quantum phase transition. Unlike a conventional thermally induced phase transition, this phase transition is driven via quantum fluctuations at zero temperature, leading to an unconventional ground state even at finite temperatures. Pressure is a representative parameter as well as carrier doping in this phase transition. At low pressures below 3-4 GPa, a piston-cylinder pressure cell (PCPC) has been most widely used for various experiments because of its large sample space. However, this pressure cell is unavailable at high pressures above 4 GPa. The CAPC marks high hydrostaticity among pressure cells available at pressures above 4 GPa. Conventionally, the CAPC has not been suitable for measurements that require a magnetic field due to its large body size. In fact, the CPAC has been used mainly for resistivity or alternating-current susceptibility and recently for neutron-scattering measurements at zero field [3].
We have fabricated the smallest PCAC to date using ϕ60 NiCrAl guide blocks and investigated pressure homogeneity from 63Cu nuclear-quadrupole-resonance (NQR) linewidth of Cu2O. The NQR frequency is proportional to the electric field gradient at a 63Cu site and the spectral shape is sensitive to local disorder or inhomogeneity. Therefore, the NQR measurements are available for investigating hydrostaticity inside the pressure cell.
Figure 1(a) shows a sectional view of the CAPC, and Fig. 1(b) shows a bird’s-eye view of three WC anvils mounted on the ϕ60 NiCrAl guide block. The four WC anvils are fitted in the NiCrAl sliding blocks covered with Teflon sheets. The four anvils move toward the 6.0-mm pyrophyllite cube without friction when the load is imposed on the NiCrAl guide blocks. The cube is made of lower and upper pyrophyllite gaskets and contains a ϕ3.0 Teflon capsule (see the inset of Fig. 2(a)). A single coil wound around Cu2O power samples is inserted into the Teflon capsule filled with pressure transmitting media, the mixture of fluorinate FC-70 and FC-77 (see Fig. 2(a)). An overview of the ϕ3.0 Teflon capsule on the gasket is shown in Fig. 2(b). The Teflon capsule has a ϕ2.5 × 1.5 mm sample space and is significantly larger than that used for conventional resistivity measurements (see the inset of Fig. 2(b)).
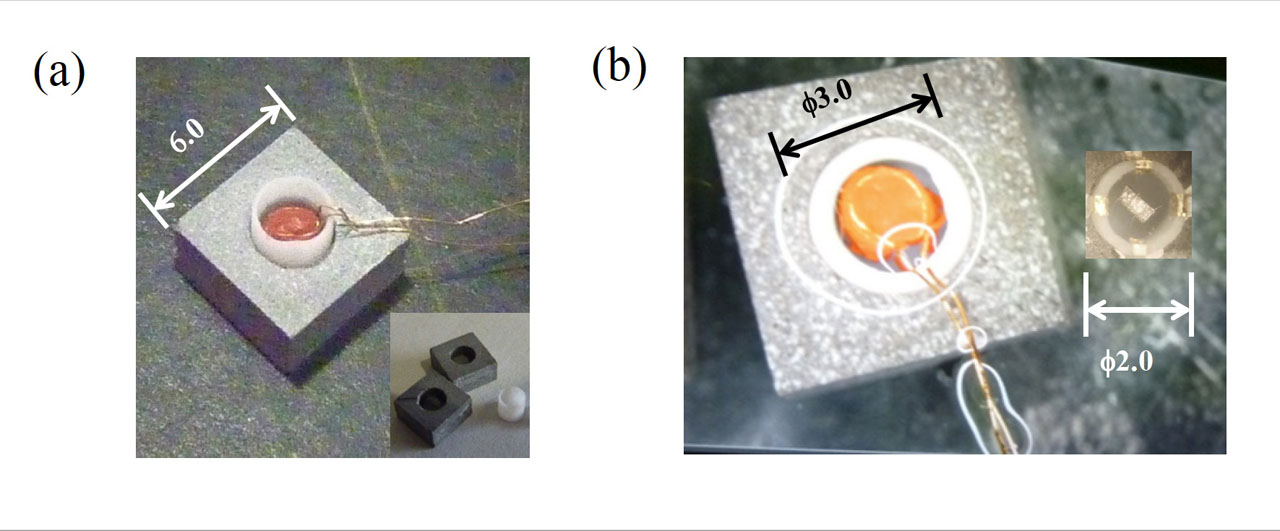
Fig. 2. (a) Bird’s-eye view of a ϕ3.0 Teflon capsule mounted on a pyrophyllite gasket. A nuclear-quadrupole-resonance (NQR) coil wrapped around powder samples of Cu2O is inserted into the capsule. The inset shows upper and lower gaskets and a Teflon capsule. (b) Overview of the ϕ3.0 Teflon capsule on the gasket. The inset shows the ϕ2.0 Teflon capsule used for the resistivity measurements.
Figure 3(a) shows the 63Cu-NQR spectra of Cu2O measured at room temperature with a conventional spectrometer and the ϕ60 CAPC. The spectra were obtained using the fast Fourier transform of the spin-echo signal. Figure 3(b) shows the pressure dependence of the NQR linewidth. The linewidth for the CAPC is compared with that for the PCPC. The NQR spectra for the PCPC exhibited double-peaks structure above 2 GPa [1], and the data below 2 GPa were plotted in Fig. 3(b). The linewidth for the PCPC increases with increasing pressure above 0.8 GPa where the mixture of the FC-70 and FC-77 begins to freeze, whereas that for the CAPC saturates at high pressures above 4 GPa. The pressure homogeneity for the CAPC is better than that for the PCPC in a high-pressure regime. This result suggests that pressure application from multiple directions suppress the inhomogeneity much more effectively than uniaxial pressure application.
In conclusion, we have fabricated a CAPC having a diameter of 60 mm, the smallest cubic-anvil cell to date, which allowed us to measure physical properties under a magnetic field utilizing a superconducting magnet with a large-bore sample space greater than 100 mm. In this pressure cell, a large sample space having ϕ2.5 × 1.5 mm was secured. This pressure cell would open a new avenue for the quest for novel pressure-induced phenomena in strongly correlated systems.
References
- [1] S. Nakagawa, J. Gochi, T. Kuwayama, S. Nagasaki, T. Takahashi, J. Cheng, Y. Uwatoko, and N. Fujiwara, Rev. Sci. Instru. 91 073907 (2020).
- [2] N. Fujiwara and Y. Uwatoko, Butsuri 76 295 (2021).
- [3] S. E. Dissanayake, M. Matsuda, K. Munakata, H. Kagi, J. Gouchi, and Y. Uwatoko, J. Phys.: Condens. Matter 31 384001 (2019).