Tuning of Luttinger Semimetal into Weyl Semimetal by Strain and Magnetic Field
Nakatsuji, Lippmaa, Katsumoto, and Kindo Groups
In the field of condensed matter physics, there has been an intense search for topological nontrivial electronic phases in strongly correlated materials. To date, most research on topological electronic systems has been limited to weakly correlated materials where electronic correlations play a minor role. Among newly-found topological phases, the Weyl semimetal phase has attracted the most significant attention because the Weyl fermions exhibit extremely high electrical and thermal conductivities, which may find use in ultrahigh-speed and low-power consumption devices. While the Weyl semimetal phase was experimentally discovered in the inversion symmetry broken TaAs system in 2015 [1,2], only a few candidates of the magnetic version have been found in systems with broken time reversal symmetry. Historically, a magnetic Weyl system is the first Weyl semimetal predicted for condensed matter in 2012 in the seminal paper by Wan et al. [3]. Notably, this prediction was specifically made for the pyrochlore iridates.
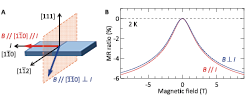
Fig. 1. Additional contribution to the negative longitudinal magnetoresistance for a Pr2Ir2O7 thin film. (A) Experimental configurations. B and I represent the external magnetic field and current, respectively. For the longitudinal (B // I) and transverse (B ⊥ I) cases, B is applied along the [110] and [110] direction, respectively. I flows along the [110] direction in both cases. (B) Magnetoresistance curves as a function of B measured at 2 K for B // I and B ⊥ I configurations. The solid and dotted lines correspond to up and down sweeps of B, respectively. The difference between the two curves comes from the chiral anomaly and indicates that the film is in the Weyl semimetal state.
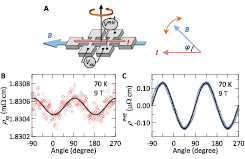
Fig. 2. Planar Hall effect in a Pr2Ir2O7 thin film. (A) Experimental configuration. I flows along the [110] direction and B is applied in the same plane. The angle between I and B (φ) is changed by the in-plane rotation of the sample. The longitudinal and planar Hall resistance is measured by Vxx and VPHE, respectively. (B and C) Angle dependence of the longitudinal and planar Hall resistivity, respectively, measured at 70 K and 9 T. The red and blue open circles are the experimental data, and the black solid lines are the fitting results using the equations predicted theoretically [10,11]. The experimental results agree well with the theory, suggesting that the film has been tuned into the Weyl semimetal state.
Here, we focus on a pyrochlore iridate Pr2Ir2O7. This material is known as a Luttinger semimetal where electronic correlations are strong [4]. According to theoretical predictions, the electronic state can be tuned into a Weyl semimetal state by perturbations such as lattice strain and external magnetic field [5,6]. However, experimental proof of the existence of the Weyl semimetal remains elusive because it is difficult to apply uniaxial strain on bulk single crystals. One possible solution to this problem is to use Pr2Ir2O7 thin films where lattice strain can be introduced through epitaxy. To that end, more than 10 groups around the world have been working on fabricating Pr2Ir2O7 thin films, but there have been no reports of success.
What makes the fabrication of the thin films difficult is the high volatility of iridium, especially at high temperatures. We therefore turned to solid-state epitaxy, namely, the deposition of precursors at room temperature by pulsed laser deposition followed by annealing in air. This synthesis route mimics bulk crystal growth and allowed us to successfully fabricate high-quality epitaxial thin films of pyrochlore Pr2Ir2O7 on yttria-stabilized zirconia substrates [7]. Detailed crystal structure analysis revealed that strained and relaxed grains coexisted in the films. When Hall measurements were performed using the Pr2Ir2O7 films, spontaneous Hall effect was found below 50 K even though the material shows no spontaneous magnetization nor was an external magnetic field applied to the film. This temperature of 50 K is very high, considering that bulk single crystals show spontaneous Hall effect only below 1.5 K [8]. The spontaneous Hall effect appears due to the all-in-all-out structure of the iridium 5d moments and the associated time reversal symmetry breaking. In this case, the theoretically predicted condition that the Weyl semimetal state appears when both the cubic symmetry and the time reversal symmetry are broken is satisfied in the strained grains in the film. On the other hand, although the relaxed grains are in the Luttinger semimetal state, it is possible that they can be tuned into a magnetic Weyl semimetal when an external magnetic field is applied and the time reversal symmetry is broken. To confirm this, we examine the effects of the chiral anomaly, which is the magneto-electric response of the Weyl semimetal. As a result, we find strong evidence for the chiral anomaly in the negative longitudinal magnetoresistance [9] (Fig. 1) and the planar Hall effect [10,11] (Fig. 2). This means that the Luttinger semimetal can be modified into the Weyl semimetal by an external magnetic field.
In summary, we have successfully fabricated epitaxial thin films of Pr2Ir2O7 and experimentally demonstrated that the Weyl semimetal state can be induced either by lattice strain or by applying an external magnetic field. Based on the results with the Luttinger semimetal, the research for the topological phases in strongly correlated systems, which has not been done so far, will proceed. It is expected to lead to the development of ultrahigh-speed and low-power devices utilizing the Weyl fermions having high electric and thermal conductivities.
References
- [1] B. Q. Lv et al., Phys. Rev. X 5, 031013 (2015).
- [2] S.-Y. Xu et al., Science 349, 613 (2015).
- [3] X. Wan et al., Phys. Rev. B 83, 205101 (2011).
- [4] B. Cheng, T. Ohtsuki et al., Nat. Commun. 8, 2097 (2017).
- [5] E.-G. Moon et al., Phys. Rev. Lett. 111, 206401 (2013).
- [6] T. Kondo et al., Nat. Commun. 6, 10042 (2015).
- [7] T. Ohtsuki et al., Proc. Natl. Acad. Sci. U.S.A. 116, 8803 (2019).
- [8] Y. Machida et al., Nature 463, 210 (2010).
- [9] D. T. Son and B. Z. Spivak, Phys. Rev. B 88, 104412 (2013).
- [10] A. A. Burkov, Phys. Rev. B 96, 041110(R) (2017).
- [11] S. Nandy et al., Phys. Rev. Lett. 119, 176804 (2017).