Interaction Effect on Adiabatic Charge Pumping via a Single-Level Quantum Dot
Kato Group
Charge transport from one reservoir to another reservoir induced by periodic operation on devices is called "charge pumping''. Recent development of device fabrication and measurement technique has enabled us to realize charge pumping in nanoscale devices such as quantum dots (QDs). In particular, quasi-static pumping under slow parameter driving, i.e., "adiabatic'' pumping is an important phenomenon because pumping charge depends only on a trajectory in the parameter space. Application of adiabatic pumping to, e.g., current standard and single electron injection has been discussed in a number of theoretical and experimental works [1].
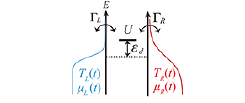
Fig. 1. Schematics of our model for adiabatic charge pumping due to driving of reservoir parameters (temperatures and electrochemical potentials). Here, εd and U are an energy level and a Coulomb interaction in a quantum dot, respectively. The dot-reservoir couplings are denoted by ΓL and ΓR.
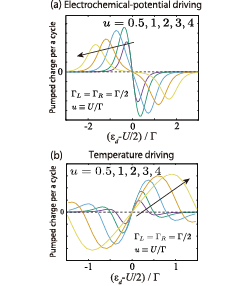
Fig. 2. Pumped charge per one cycle obtained by the renormalized perturbation theory for (a) the electrochemical-potential driving case and (b) the temperature-driving case.
For adiabatic pumping, we need to take a period of driving as larger than characteristic time scales of the system. Therefore, coherent transport in quantum dots strongly coupled with electronic leads, which have a short relaxation time, is suitable to fast adiabatic pumping. However, theoretical framework for charge pumping in QDs strongly mixed with leads has not been studied so far. In our previous paper [2], we calculated pumping charge within the first-order perturbation theory with respect to Coulomb interaction. In the present study [3], we aimed to construct general formalism, which can treat arbitrary strength of Coulomb interaction beyond our previous work.
We considered adiabatic charge pumping via a single-level QD system induced by reservoir parameter driving in the coherent transport region (see Fig. 1). We formulated pumped charge for arbitrary Coulomb interaction by nonequilibrium Green’s function method. To handle the time-dependent reservoir temperatures, we introduced a thermomechanical field, which describes temperature modulation via an energy rescaling of the reservoirs. We also employed the adiabatic approximation to obtain pumped charge under adiabatic operation.
We derived a general formula for the pumped charge in terms of the Berry connection. It is expressed by the two-particle Green’s function of electrons in the QD. Our formalism covers the low-temperature strongly-correlated region, which cannot be treated in the previous theoretical methods. Based on our general formulism, we calculated the pumped charge by employing the renormalized perturbation theory (see Fig. 2). We showed that for both the electrochemical-potential-driven and the temperature-driven case, the pumping strength has a maximum and a minimum as a function of the QD energy level, and the position of the maximum (minimum) moves away from the particle-hole symmetric point as the Coulomb interaction increases. For the electrochemical-potential-driven case, the maximum value of the pumping strength is once enhanced, and then suppressed as the Coulomb interaction increases (see Fig. 2 (a)). On the other hand, for the temperature-driven case, the maximum value continues to increases as the Coulomb interaction increases (see Fig. 2 (b)). This difference was explained from the renormalized parameters consistently.
Our formalism states that adiabatic charge pumping can be evaluated by the two-particle Green’s function of the electrons in the QD, which is in principle calculated by numerical methods. It is an important future problem to compute the pumped charge without any approximation in the strong Coulomb interaction region. From the viewpoint of non-equilibrium thermodynamics, it is also a challenge to generalize the present formalism to heat pumping and work exchange and to discuss quantum effects on efficiency of small engines.
References
- [1] J. P. Pekola et al., Rev. Mod. Phys. 85, 1421 (2013).
- [2] M. Hasegawa and T. Kato, J. Phys. Soc. Jpn. 86, 024710 (2017).
- [3] M. Hasegawa and T. Kato, J. Phys. Soc. Jpn. 87, 044709 (2018).