Magnetic Fields up to 370 T Revealed Aharonov-Bohm Exciton Splitting in Carbon Nanotubes
Takeyama Group
Rolled graphene with a certain chirality vector (n, m) forms a single-walled carbon nanotube (SWNT), possessing different electrical properties depending on the chirality, changing from semiconductors to metals. The penetration of magnetic flux quanta into a SWNT breaks the time-reversal symmetry of the electronic states, and the effect is known as the Aharonov-Bohm (A-B) effect. The quasi-one dimensionality of SWNTs enhances the effects of exciton as well as electron correlation in the band-edge optical properties. Owing to the A-B effect, a magnetic flux penetrating the cross section of a SWNT induces the valley splitting between the K and K’ points in the Brillouin zone, along with a mixing of the exciton wave functions, and eventually the bright exciton and its dark counter part are converted into two independent bright K and K’ point excitons in a limit of strong magnetic fields. The k · p theory by Ando [1], has predicted magnetic field evolution of the K and K’ point exciton absorption spectra differently, depending on the relative energy-level ordering of the bright and dark band-edge excitons.
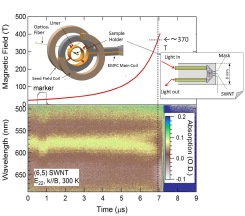
Fig. 1. A streak 2-dimensional image of the E22 second sub-band absorption of (6,5) SWNTs with a signal of magnetic field measured at 300 K. The inset illustrates sample setting with optical fibers inside a EMFC coil.
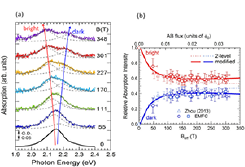
Fig. 2. (a) The band-edge absorption spectra at each magnetic field obtained from Fig.1. (b) The absorption intensity evolution in magnetic fields. The solid lines are the results of fitting using a two-energy level model (dotted line) modified with a linear B term. Triangles are obtained by the single-turn coil ultra-high magnetic field generator in magnetic fields up to 160 T.
We have observed a discernible linear peak splitting of the K and K’ point exciton absorption spectra of SWNTs in magagauss magnetic fields above the field where the exciton wave function mixing is over. Highly-selected (6,5) single-chirality SWNTs synthesized by a single-surfactant multicolumn-gel-chromatography method [2] were further processed to highly alignment of the tubes to yield average 29 degrees alignment by a polyvinyl alcohol thin film stretching.
The thin stretched film sample was set inside of the flux-compression coil with input and output optical fibers in such a way that magnetic field was oriented along the film stretching direction (the Voigt configuration). The transmission light was detected by a streak camera equipped with a CCD camera. A streak image of absorption spectra was obtained in magnetic fields up to around 370 T generated by the electro-magnetic flux compression ultra-high magnetic field generator, and the result was shown for the E22 second sub-band transition of a sample of (6,5) chirality SWNTs in Fig. 1. Absorption peak centered at 575 nm showed clearly a splitting when the magnetic field exceeds 170 T. The absorption spectra were plotted at each magnetic field strength from Fig. 1, and plotted in Fig. 2(a) with those of Lorentzian de-convolution spectral lines (dotted line). The peak energy splits linearly with magnetic field with the splitting Δab = μBeff (where, μ: exciton splitting coefficient = 0.5, Beff = Bcos(29), and B is the strength of an external magnetic field) above 150 T. The integrated intensity of the absorption spectrum of each K and K’ splitting component exhibited linear magnetic field dependence above 200 T, but with their intensity recovers to that of the original peak, respectively, as seen in Fig. 2(b). Simple two-energy level mixing model does not hold anymore [3], but should be modified with a linear B term. All these phenomena evolve quite similarly with those presented by the theory of T. Ando [1]. We have figured out that the linear term is correlated to the environmental static dielectric constant, and found that the value amounts to 50, which arises likely from residual water molecules in hydrophilic PVA film [4].
References
- [1] T. Ando, J. Phys. Soc. Jpn. 75, 024707 (2006).
- [2] H. Liu et al., Nat. Commun. 2, 309 (2011).
- [3] W. Zhou, D. Nakamura, H. Liu, H. Kataura, and S. Takeyama, Scientific Reports 4, 6999 (2014).
- [4] D. Nakamura, T. Sasaki, W. Zhou, H. Liu, H. Kataura, and S. Takeyama, Phys. Rev. B 91,235427 (2015).