Discovery of a New Magnetic Material: “Weyl Magnet”
S. Nakatsuji, T. Kondo, and S. Shin
In 2015, Weyl fermions have been discovered for the first time near the Fermi level in the non-magnetic semimetal TaAs. Weyl points in the momentum space serve as a pair of magnetic monopoles through the topological aspects of the wavefunctions for electrons [1]. Moreover, the fictitious magnetic fields due to the monopoles may induce novel electric transports, and could be useful for low energy consumption electronics. In contrast to the non-magnetic Weyl fermions in TaAs, magnetic Weyl fermions are known to appear in magnets, thus would enable us to control Weyl fermions by external magnetic field. This functionality will be necessary for device applications, and many efforts have been made for searching magnetic Weyl fermions. However, they have remained hypothetical so far.
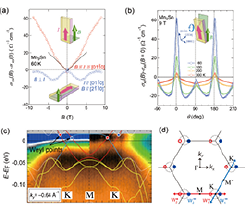
Fig. 1. Evidence for Weyl fermions in Mn3Sn:(a)Weyl fermions in condensed matter systems show an unconventional positive longitudinal magnetoconductivity (negative longitudinal magnetoresistivity), called “chiral anomaly”. (b) This appears only when magnetic field is applied parallel to electric field (B//I, θ = 0 deg.). With an increasing magnetic field, the breaking of the imbalanced charge conservation between the Weyl points with opposite chirality makes the materials more conductive. This novel phenomenon is known as one of the experimental evidence of the Weyl fermions. (c) ARPES band mapping near the Fermi level is compared with DFT band calculations. Weyl (band crossing) points with opposite chirality are denoted by blue and red closed circles, respectively. (d) These Weyl points are found along the K–M–K line in the bands on the kz = 0 plane near EF.
Recently, an antiferromagnetic manganese-tin alloy Mn3Sn is found to exhibit a large anomalous Hall and Nernst effects, even at room temperature [2, 3]. Usually, these anomalous Hall and Nernst effects are known to be proportional to magnetization and thus have been observed only in ferromagnets. The spontaneous Hall resistivity in the antiferromagnet with vanishingly small magnetization indicates that the large fictitious field equivalent to a few hundred T must exist in the momentum space. Recent DFT calculation predicts that the large fictitious field or Berry curvature may well appear due to the formation of Weyl points nearby the Fermi energy EF [4].
Nakatsuji, Kondo and Shin groups at ISSP University of Tokyo and their theoretical collaborators at RIKEN have demonstrated the realization of magnetic Weyl fermions in Mn3Sn for the first time. Our study has revealed the existence of a “Weyl magnet”, a new magnet with tunable magnetic Weyl fermions by magnetic fields at room temperature [5]. We found strong experimental evidence for the Weyl fermions in Mn3Sn, namely, that the band structure revealed by angle resolved photoemission spectroscopy (ARPES) is found roughly consistent with density functional theory (DFT) and the chiral anomaly is clarified in the magnetotransport measurements (Fig. 1). Thus, these experiments demonstrate that the large anomalous Nernst signals arise from the Berry curvature associated with the Weyl points near the Fermi energy.
Our groups have revealed extremely large magnetic transports and thermoelectric effects in the Mn3Sn magnet. By their new discovery of Weyl magnet, the mystery of these novel properties would be solved. We anticipate that further new phenomena will emerge through the interplay between electron correlation and topology in Weyl magnets.
References
- [1] B. Q. Lv, H. M. Weng, B. B. Fu, X. P. Wang, H. Miao, J. Ma, P. Richard, X. C. Huang, L. X. Zhao, G. F. Chen, Z. Fang, X. Dai, T. Qian, and H. Ding, Phys. Rev. X 5, 031013 (2015).
- [2] S. Nakatsuji, N. Kiyohara, and T. Higo, Nature 527, 212 (2015).
- [3] M. Ikhlas, T. Tomita, T. Koretsune, M. –T. Suzuki, D. Nishio-Hamane, R. Arita, Y. Otani, and S. Nakatsuji. Nature Physics 13, 1085 (2017).
- [4] H. Yang, Y. Sun, Y. Zhang, W-J. Shi, S. S. P. Parkin, and B. Yan, New J. Phys. 19, 015008 (2017).
- [5] K. Kuroda, T. Tomita, M.-T. Suzuki, M.-T. Suzuki, C. Bareille, A. A. Nugroho, P. Goswami, M. Ochi, M. Ikhlas, M. Nakayama, S. Akebi, R. Noguchi, R. Ishii, N. Inami, K. Ono, K. Kumigashira, A. Varykhalov, T. Muro, T. Koretsune, R. Arita, S. Shin, T. Kondo, and S. Nakatsuji, Nature Materials 16, 1090 (2017).