Large Magneto-Optical Kerr Effect and Imaging of Magnetic Octupole Domains in the Non-Collinear Antiferromagnetic Metal Mn3Sn
Nakatsuji Group
When a polarized light beam is incident upon the surface of a magnetic material, the reflected light undergoes a polarization rotation. This magneto-optical Kerr effect (MOKE) has been intensively studied in a variety of ferro- and ferrimagnetic materials because it provides a powerful probe for electronic and magnetic properties as well as for various applications including magneto-optical recording. Recently, there has been a surge of interest in antiferromagnets (AFMs) as prospective spintronic materials for high-density and ultrafast memory devices, owing to their vanishingly small stray field and orders of magnitude faster spin dynamics compared to their ferromagnetic counterparts [1]. In fact, the MOKE has proven useful for the study and application of the antiferromagnetic (AF) state. Although limited to insulators, certain types of AFMs are known to exhibit a large MOKE, as they are weak ferromagnets due to canting of the otherwise collinear spin structure [2]. In the fully compensated collinear AFMs, where the MOKE is usually absent, quadratic magneto-optical effects such as the Voigt effect can be useful to determine the Néel vector [3]. On the other hand, recent theoretical and experimental progress has revealed that systems such as certain spin liquids and non-collinear AFMs can exhibit a large anomalous Hall effect (AHE) in zero applied magnetic field despite a vanishing magnetization [4, 5]. Because the AHE has the same symmetry requirements as the MOKE [6], it is possible that the same class of AFMs may exhibit a Kerr rotation. Thus, the recent experimental discovery of a large AHE in the non-collinear AFM Mn3Sn [5], which is recently reported to be the first example of a Weyl magnet [7], as well as its soft response to a magnetic field give promise for a potentially large MOKE character.
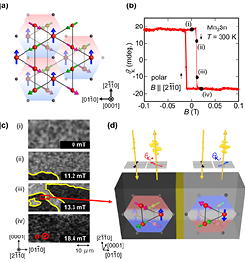
Fig. 1. (a) Cluster magnetic octupole ordering of Mn3Sn. Large red (small dark grey) and large transparent orange (small transparent grey) spheres represent Mn (Sn) atoms forming kagome planes at z = 0 and 1/2, respectively. The Mn magnetic moments (arrows) lie in the (0001) plane and form an inverse triangular spin structure. Different colored arrows represent three sublattices. The spin structure on the kagome bilayers can be considered as ferroic order of cluster magnetic octupoles. (b) Field B dependence of the polar magneto-optical Kerr rotation angle θK for the (2110) plane in B || [2110]. (c) Evolution of the antiferromagnetic domains of the (2110) plane as a function of B || [2110] (−21 mT ≤ B ≤ 21 mT). The imaging area is 25 μm × 50 μm. Grey and black regions correspond to positive and negative values of the MOKE signal. (d) Schematic illustration of two regions with different MOKE image contrasts (grey/black areas) due to opposite signs of the Kerr angles θK,−/+, corresponding to two types of cluster magnetic octupole domains that have inverse triangular spin structures with opposite spin directions within the (0001) plane. The two regions should be separated by a domain wall (yellow area).
Mn3Sn is a hexagonal antiferromagnet (space group: P63/mmc), which has the ABAB sequence of the Mn kagome layer along [0001] and exhibits the non-collinear AF spin structure below the Néel temperature of TN ~ 430 K [4]. This three-sublattice AF state on the kagome bilayers can be viewed as “ferroic ordering of cluster magnetic octupoles” (Fig. 1(a)) [8]. In addition to this dominant order parameter, the moments cant slightly in the plane to produce a small net ferromagnetic (FM) moment of ~0.002 μB/Mn within the (0001) plane. Although the subdominant FM order is not responsible for the AHE [4] (and MOKE as we discuss below), it is essential (together with concomitant weak in-plane anisotropy) for magnetic field to control the AF spin structure. This is demonstrated, for example, by the sign reversal of the AHE using a small applied field of B ~ 15mT within the (0001) plane, corresponding to the in-plane rotation of the Mn moments.
We carried out field-swept measurements of the polar MOKE using the Mn3Sn (2110) plane with a λ = 660 nm linearly polarized laser at 300 K. Significantly, a large zero-field Kerr rotation angle θK ~ 20 mdeg and a clear square hysteresis loop are observed in B || [2110] (Fig. 1(b)), which is comparable to the case for ferromagnetic materials. The coercive field BC ~ 12 mT is consistent with the hysteresis curve obtained in ρH(B), indicating that the magnetic properties at the surface are nominally identical to those in the bulk [4]. Moreover, our polar MOKE spectroscopy and first-principles calculation for the frequency dependence of the Kerr rotation θK(ω) have clarified that ferroic ordering of magnetic octupoles in the non-collinear Néel state may cause a large MOKE even in its fully compensated AF state. The large MOKE signal without spin magnetization sharply contrasts with the previously known AF insulator cases where weak ferromagnetism has been believed to be essential for the presence of the MOKE. This large MOKE further allows imaging of the magnetic octupole domains and their reversal induced by B. Figure 1(c) presents a series of the polar MOKE images of the Mn3Sn (2110) plane under B || [2110] (− 21 mT ≤ B ≤ 21 mT) which obtained at the points (i)-(iv) in Fig. 1(b). Here, grey and black colors correspond to positive and negative values of the polar MOKE signal parallel to the [2110] direction originating from the two types of the cluster magnetic octupole domain in Fig. 1(d). This is the first observation of domain reversal in an AF metal and of magnetic octupole domain reversal by MOKE microscopy [7]. The large MOKE observed in an AF metal should open new avenues for the study of domain dynamics as well as spintronics using AFMs.
References
- [1] T. Jungwirth et al., Nat. Nanotech. 5, 231 (2016).
- [2] F. J. Kahn et al., Phys. Rev. 186, 891 (1969).
- [3] V. Saidl et al., Nat. Photon. 11, 91 (2017).
- [4] Y. Machida et al., Nature 463, 210 (2010); H. Chen et al., Phys. Rev. Lett. 112, 017205 (2014); N. Kiyohara, T. Tomita, and S. Nakatsuji, Phys. Rev. Applied 5, 064009 (2016).
- [5] S. Nakatsuji, N. Kiyohara, and T. Higo, Nature 527, 212 (2015).
- [6] W. Feng et al., Phys. Rev. B 92, 144426 (2015).
- [7] K. Kuroda and T. Tomita et al., Nat.Mater. 16, 1090 (2017).
- [8] T. Higo, H. Man, D. Gopman, L. Wu, T. Koretsune, O. van ’t Erve, Y. Kabanov, D. Rees, Y. Li, M.-T. Suzuki, S. Patankar, M. Ikhlas, C. L. Chien, R. Arita, R. Shull, J. Orenstein, and S. Nakatsuji, Nat. Photon. 12, 73 (2018).