Multiband Superconductivity with Unexpected Deficiency of Nodal Quasiparticles in CeCu2Si2
Sakakibara Group
The gap symmetry of unconventional superconductors has attracted much attention of scientific community because it is closely related to the exotic pairing mechanism. CeCu2Si2 (Tc ~ 0.6 K) is a historically important compound that made a breakthrough in the field of superconductivity; in 1979, exotic superconductivity was discovered for the first time in this strongly-correlated heavy-electron system [1]. Until quite recently, its gap symmetry was believed to be a nodal d-wave pairing mediated by spin fluctuations [2]. The presence of line nodes had been suggested from the power-law temperature dependence of various physical quantities, such as the NMR relaxation rate 1/T1 [3] and the specific heat, both of which were measured in the intermediate temperature region above 0.1 K. Recent interest has been focused on the location of line nodes: whether the gap symmetry is dx2−y2 or dxy type [4,5].
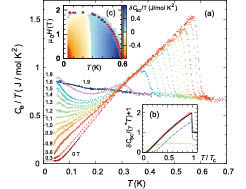
Fig. 1. (a) Temperature dependence of the electronic specific heat of CeCu2Si2 divided by temperature, Ce /T, for H // [100]. (b) Temperature dependence of Ce /T at zero field, where the T-dependent normal-state contribution is subtracted. The solid line is a best fit to the two-gap BCS model. (c) Field-temperature phase diagram for H // [100] and a contour plot of the superconducting contribution to Ce /T. Anomalous behavior that can be ascribed to the Pauli paramagnetic effect is clearly seen in the high-field and low-temperature region below 0.1 K.
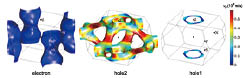
Fig. 2. The calculated Fermi surfaces of CeCu2Si2 colored by the magnitude of the Fermi velocity νF.
To elucidate the gap symmetry of CeCu2Si2, we performed specific-heat measurements at low temperatures down to 40 mK using a high-quality single crystal (S-type)[6]. Quite unexpectedly, the zero-field specific heat Ce exhibits exponential temperature dependence below 80 mK (Fig. 1(a)), reminiscent of full-gap superconductivity. In addition, we provide thermodynamic evidence for nodeless superconductivity that the low-temperature specific heat is proportional to the magnetic field at low fields for all field orientations and does not change with the c-plane field rotation. We found that the temperature dependence of the zero-field Ce /T including the linear behavior in the intermediate-T region can be reproduced on the basis of a phenomenological two-gap model within the conventional BCS framework using two BCS gaps, Δ1 = 1.76kBTc and Δ2 = 0.7kBTc, whose weights are 65% and 35% of the total density of states, respectively (Fig. 1(b)). We also found anomalous behaviors in the field variations of the specific heat as well as the magnetization at low temperatures in the high-field regime (Fig. 1(c)), which can be interpreted as a strong Pauli paramagnetic effect occurring in a multiband superconductor. All the present results match with the prediction of multiband superconductivity in the absence of nodal quasiparticles.
One may suspect that line nodes might exist in the gap on light-mass bands, since the specific heat measurement mainly probes the heavy-mass band. To get an insight into the band structure of CeCu2Si2, we have performed first-principles calculations by the LDA+U method. As shown in Fig. 2, CeCu2Si2 has a flat electron band around the X point with the heaviest mass and two hole bands around the Z point. In this Fermi-surface topology, the deficiency of nodal quasiparticles requires the negligibly small effective mass of the two light-mass bands, which contradicts the multiband feature detected from the specific-heat measurement. This leads to a conclusion that the pairing symmetry of CeCu2Si2 is not the anticipated d-wave state, but the multiband full-gap state, including an unconventional s-wave such as s±-wave, a conventional s-wave, or a fully-gapped d+id state. These findings would open a new door into electron pairing in CeCu2Si2 and help understand the pairing mechanism of exotic superconductors.
References
- [1] F. Steglich et al., Phys. Rev. Lett. 43, 1892 (1979).
- [2] O. Stockert et al., Nat. Phys. 7, 119 (2011).
- [3] Y. Kitaoka et al., J. Phys. Soc. Jpn. 55, 723 (1986).
- [4] H. A. Vieyra et al., Phys. Rev. Lett. 106, 207001 (2011).
- [5] I. Eremin et al., Phys. Rev. Lett. 101, 187001 (2008).
- [6] S. Kittaka et al., Phys. Rev. Lett. 112, 067002 (2014).