Relaxor Behavior and Morphotropic Phase Boundary in a Simple Model
Kato Group
Ferroelectric relaxors made from perovskite oxides (ABO3) have attracted much interest because of their characteristic dielectric properties suitable for application. The common feature of perovskite-type relaxors is intrinsic randomness due to compositional disorder. It is well known that structural phase transition induced by change in composition plays a special role for obtaining excellent dielectric properties; the region near this phase transiton is called the morphotropic phase boundary (MPB).
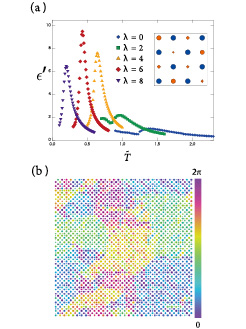
Fig. 1. (a) Plot of real part of dielectric susceptibilities per site. Data are horizontally shifted for each value of λ. (Inset) Blue and red circles indicate A- and B-sublattice on a square lattice. (b) A snapshot of dipole configurations for λ=6. Sizes of radii represent sizes of projected dipole moments, and colors of circles indicate directions of dipole moments.
The origin of large dielectric response at the MPB was discussed within the Landau-Ginzburg-Devonshire (LGD) theory. The LGD theory is, however, not satisfactory to describe the whole properties of relaxors since it treats only spatially-averaged quantities. In particular, important information on spatial profiles of electric polarization such as domain structure and spatial inhomogeneity due to compositional disorder cannot be discussed by the LGD theory.
In order to understand physics near the MPB, we have performed large-scale Monte Carlo simulation [1] to a simplified dipole model on a square lattice given as
Here, μi is a three-dimensional vector representing an electric polarization caused by the ionic displacement at site i, and rij is a displacement vector from site i to j. In order to represent intrinsic compositional randomness at B-site in perovskite oxides, we divide the square lattice into two sub-lattices, A and B as shown in the inset of Fig. 1(a), and assume that magnitude of the A-site dipole moments is fixed as unity (μA =1), whereas that of the B-site dipole moments has spatial distribution. The magnitude of the B-site moment is chosen randomly as μB = max(0.8-0.1n,0), where n is an integer-valued random variable following the Poisson distribution P(n) = λn exp(-λ)/n!. We have performed Monte Carlo calculation by using efficient O(N) algorithm optimized for long-rage interaction [2].
In Fig. 1(a), we show real part of calculated dielectric susceptibilities as a function of the temperature T. The peak of the dielectric susceptibility is well suppressed for small λ, whereas it rapidly grows with increasing λ. The maximum value becomes largest at an optimum value λ=5-6, and is reduced for larger value of λ. These features well resemble the behavior of the dielectric response near MPB in perovskite oxides, identifying λ=5-6 to be the MPB.
To examine mechanism of emerging of the maximum, a snapshot at λ=6 is shown in Fig. 1(b). We observe meso-scale ferroelectric domain, whose size becomes significantly large near λ=5-6. This remarkable enhancement of correlation length for ferroelectric ordering, indicated from the snapshot, is due to phase competition. For small λ, anti-ferroelectric phase is stabilized at low temperatures, whereas relaxor ferroelectric phase is realized for large λ. Direction of polarization is diagonal in the former phase, and is along neighboring sites in the latter phase. As a result, the easy-axis potential is mixture of the ones of these two phases near the MPB (λ=5-6). The resulting mixed potential forms the dimple at the bottom of a wine bottle, and it makes dipoles easy to rotate. This result indicates that local polarization rotation under suppressed anisotropy makes domain wall flexible to external field, leading to huge dielectric response.
In summary, we proposed a simple dipole model, and executed Monte Carlo simulations to it. We showed that there appears a boundary ferroelectric phase between two phases, and that it has large ferroelectric domains with flexible walls. Our result near the MPB can be related to recent experiments by neutron scattering [3] and transmission electron microscopy [4].
References
- [1] Y. Tomita and T. Kato, J. Phys. Soc. Jpn. 82, 063002 (2013).
- [2] K. Fukui and S. Todo, J. Comp. Phys. 228, 2629 (2009).
- [3] M. Matsuura, K. Hirota, P. M. Gehring, Z.-G. Ye, W. Chen, and G. Shirane, Phys. Rev. B 74, 144107 (2006).
- [4] K. Kurushima and S. Mori, Materials Science and Engineering 18, 092015 (2011).